Historic Results
Experimental physicists at Fermilab are constantly at work systematically building our understanding of the laws of nature. The following are some of the hallmark discoveries they have made at the three frontiers of research in particle physics.
Energy Frontier
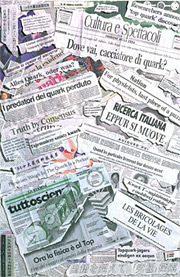
Discovery of the Top Quark
Physicists observed the first proton-antiproton collisions produced by the Tevatron on Oct. 13, 1985. Researchers at the CDF experiment and at DZero, which began operating later in 1992, used the Tevatron to study matter at ever smaller scales.
On March 2, 1995, physicists at CDF and DZero announced the discovery of the top quark. Researchers in both collaborations had statistically proven observation of the top quark in collisions at their detectors.
The top quark, which is as heavy as a gold atom but much smaller than a proton, was the last undiscovered quark of the six predicted to exist by current scientific theory. Scientists worldwide had sought the top quark since the discovery of the bottom quark at Fermilab through fixed-target experiments in 1977.
Both collaborations were subsequently able to measure the mass of the top quark to high precision. Particle physicists measure particle masses to verify their particle models. Knowing the value of the top quark mass has allowed physicists to zero in on the mass of the undiscovered Higgs boson, a crucial component of the theoretical framework of particle physics.
Discovery of the Bottom Quark
In 1977, an experiment led by physicist and Nobel laureate Leon Lederman at Fermilab provided the first evidence for the existence of the bottom quark, an essential ingredient in the theoretical framework called the Standard Model.
Using a fixed-target experiment, the collaboration discovered a particle, which they called an upsilon. It was composed of a previously unobserved kind of quark, the bottom quark, and its antimatter partner, the antibottom quark. The bottom quark was then the heaviest sub-nuclear particle ever observed, weighing in at 10 times the mass of a proton.
Physicists at Brookhaven National Laboratory and SLAC National Accelerator Laboratory had in 1974 discovered particles made up of charm quarks and their antimatter partners, anticharm quarks. The discovery of the bottom quark provided important proof of the speculation that all matter is made up of quarks.
Click here to read the original press release.
Constant Advances
Researchers from CDF and DZero reported new results every week in the Result of the Week column of Fermilab’s daily newsletter, Fermilab Today.
Intensity Frontier
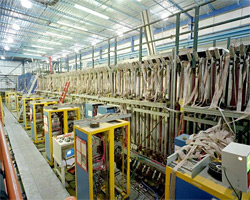
CP Violation in Kaons
When certain subatomic particles called kaons decay, they break into a charged pion, a neutrino and either an electron or its antimatter counterpart, a positron. In the absence of CP violation, the number of electrons and positrons created in these decays would be equal. However, scientists have observed that the scales tip slightly toward decay into electrons. This provides proof that CP violation can lead to an excess of matter over antimatter. If this process occurred in the early universe, all of the positrons would annihilate upon encountering electrons. But after all of the positrons had disappeared, some matter would remain.
This result gives credence to the theory that CP violation allowed all of us to exist. But the effects of the process that causes an excess of matter from kaon decays are too small to complete the picture. The observed difference is orders of magnitude away from explaining asymmetry in the universe. This is one of the reasons that scientists are so interested in observing CP violation in other places, like neutrinos.
Observation of Tau Neutrino
The Nu Tau or DONUT collaboration at Fermilab announced on July 21, 2000, the first direct evidence for the subatomic particle called the tau neutrino, the third kind of neutrino known to particle physicists.
Although earlier experiments had produced convincing indirect evidence for the particle’s existence, no one had directly observed a tau neutrino, an almost massless particle carrying no electric charge and barely interacting with surrounding matter.
The collaboration reported 12 instances of a neutrino interacting with an atomic nucleus to produce a charged particle called a tau lepton, the signature of a tau neutrino. To make this find, they aimed Fermilab’s intense beam of neutrinos across a 3-foot-long target of iron plates sandwiched with emulsion, similar to photographic film, which recorded the particle interactions. In the target, one out of 1 trillion tau neutrinos interacted with an iron nucleus and produced a tau lepton, which left its 1-millimeter-long tell-tale track in the emulsion. Physicists needed about three years of painstaking work to identify the tracks revealing a tau lepton and its decay, the key to exposing the tau neutrino’s secret existence.
Unexpected Behavior of Neutrinos
Experimenters at Fermilab’s NuTeV, Neutrinos at the Tevatron, experiment discovered an imbalance of neutrinos and muons emerging from high-energy collisions of neutrinos with target nuclei in a 700-ton detector.
The results of generations of particle experiments with other particles have yielded precise predictions for the value of this ratio, which characterizes the interactions of particles with the weak force, one of the four fundamental forces of nature. But neutrinos did not fall into line with those expectations.
Experimenters using the Large Electron Positron at CERN, the European particle physics laboratory, measured the same neutrino interaction in a different particle reaction. They saw the same discrepancy, although with less precision. If the discrepancy is real, it could be another indication that neutrinos truly are different.
Cosmic Frontier
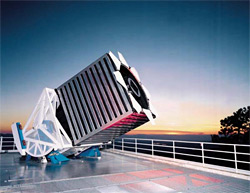
Origins of High-Energy Cosmic Rays
In 1993, Fermilab physicists proposed the construction of the world’s largest cosmic ray detector, the Pierre Auger Observatory, to address the question of the origin of high-energy cosmic rays. Researchers previously had assumed that cosmic rays approach the Earth uniformly from random directions. However, in 2007, researchers at Pierre Auger announced that the most energetic cosmic rays to impact the Earth generally come from the direction of active galactic nuclei.
Many large galaxies, including our own Milky Way, has a supermassive black hole in its center. While most black holes will sit quietly in the nucleus of a galaxy for billions of years, if a galaxy’s black hole happens to be surrounded and fed by a steady stream of gas and stars, it creates what is called an active galactic nucleus. An active galactic nucleus releases high-energy radiation observable by radio, x-ray and gamma ray telescopes on earth.
Defining the Horizon
Outer space is not empty. For example, on average, one electron floats in every cubic centimeter of space between the planets and stars in our solar system. Between the galaxies, one can find a diffuse sea of photons and other particles. When cosmic rays travel through this medium, they sometimes interact with these particles.
When cosmic rays with high energies collide into other particles, they recoil and lose a fraction of their energy. Lower energy cosmic rays can pass through these particles without interacting. The farther a cosmic ray travels through space, the greater chance it has of running into one of these particles.
Based on this information, scientists hypothesized that they would observe more low-energy cosmic rays than expected and fewer high-energy cosmic rays, since the high-energy cosmic rays would break down into lower-energy cosmic rays as they moved through space.
Detectors at the Pierre Auger Observatory confirmed this theoretical prediction. Because researchers know that high-energy cosmic rays cannot travel too far through space before colliding with something, they can set limits on how far away the source of a high-energy cosmic ray that makes it to the Earth can be. This helps astrophysicists decide where to look in space for the source of high-energy cosmic rays.
Confirming Evidence of Dark Energy
Scientists found the first evidence of dark energy in 1998 when they discovered through the observation of distant supernovae that the universe was expanding at an increasing rate. They had expected to find a slowing the rate of expansion due to the force of gravity. The observation to the contrary led them to theorize that another force was pushing the universe apart.
Fermilab researchers found a way to test the finding with the Sloan Digital Sky Survey. Through SDSS, scientists could observe large clusters of galaxies and connect them to fluctuations in the cosmic microwave background, mapped by a separate satellite called WMAP. The cosmic microwave background gives astrophysicists a picture of the universe as it was about 300,000 years after the big bang. Objects in space have left imprints on the cosmic microwave background in the form of areas of concentrated particles and energy. Imagine those hot spots as the light areas left on an old carpet when a sofa that has sat in one place for years is put in another room. SDSS measured the deflection light from the background hotspots, or the light spots on the carpet, as it passed by the foreground galaxy clusters, or furniture. From this, researchers deduced that the accelerating expansion of the universe was real.
Scientists compared the intensities of the hot spots left in the cosmic microwave background to the locations and sizes of clusters of galaxies they observed with the SDSS to determine how the universe has expanded since a time shortly after the big bang until the present epoch. The experiment confirmed that the universe has expanded at an increasing rate. This offered independent confirmation of the study that suggested the existence of dark energy.
- Last modified
- 04/28/2014
- email Fermilab